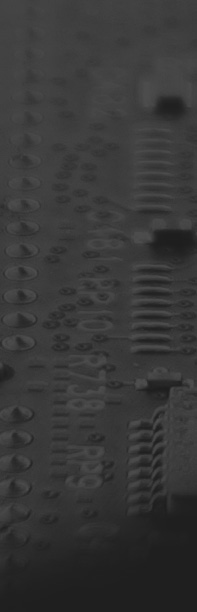
Making Stuff: Smaller
Future technologies will depend on tiny stuff—from silicon chips to micro-robots that probe the human body. Airing August 21, 2013 at 9 pm on PBS Aired August 21, 2013 on PBS
- Originally aired 01.26.11
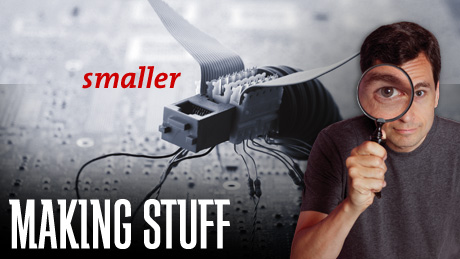
Program Description
Transcript
Making Stuff: Smaller
PBS Airdate: January 26, 2011
DAVID POGUE: Imagine a world with buildings that can ride out earthquakes; bacteria that make gasoline; tiny devices that can repair individual cells, or even D.N.A.; gossamer threads, strong enough to hold up a bridge; or an elevator to the stars. These visions of the future are based in the discoveries of today, as a new science of materials emerges from the elemental building blocks of the universe, promising a future in which we can create virtually anything we want, atom by atom.
I'm David Pogue, and I'm on a quest to discover how the world's smallest materials are changing our lives: swarms of nano-machines that combat cancer on the cellular level with bee venom;...
Bee venom is a cancer drug?
Samuel Wickline (Cardiologist, Washington University): Yeah, it's an excellent cancer drug.
DAVID POGUE: ...computer chips, one-atom-thick and up to a thousand times faster than silicon chips today;...
Come on; now you're hurting my brain.
Daniel S. Mishkin (Gastroenterologist, Boston University): This capsule is acquiring images at a rate of two frames per second.
DAVID POGUE: ...and high definition cameras, an inch long, monitoring our bodies from within.
Oh man, I think I just banged the top of the eyeball.
How far can we go...
What? That's a robot?
Bradley Nelson (Institute of Robotics, ETH Zurich): That's a robot.
DAVID POGUE: ...in Making Stuff Smaller? Right now, on Nova.
As tech columnist for the New York Times, I'm always on the hunt for the next killer gadget.
Wow, so I can play my records straight into the iPhone?
Adam Cohen (Consumer Electronics Show participant): That's right.
DAVID POGUE: That's very cool.
Each year, my beat brings me to the Consumer Electronics Show, in Las Vegas, to investigate the latest trends in new tech.
Summer Douglass (Consumer Electronics Show participant): It's a watch that is also a cellular phone, an Mp3 player, a camera, a video camera.
DAVID POGUE: Somehow, the electronics world has found that the key to making stuff better is making it smaller.
David Albers (Consumer Electronics Show participant): So, that's the new flagship L.E.D.
DAVID POGUE: Wow.
Take the T.V. Ten years ago, the first flat screens cost as much as $12,000. They had thick glass panels and weighed a whopping 85 pounds, but now?
DAVID ALBERS: This T.V. is point-three inches deep.
DAVID POGUE: Are you kidding? So, the nice thing is when they FedEx it to you, they can just slip it under the door?
DAVID ALBERS: Exactly.
DAVID POGUE: I'm on a quest to discover why size matters, why getting smaller leads to such gigantic benefits.
Amy Koppman (Consumer Electronics Show participant): Performance on it is phenomenal in the light. You have an f2.8 lens, 24-millimeter wide. It's just a great piece.
DAVID POGUE: It's crazy. If everything we own had improved over the last 25 years as much as electronics have, the average family car would travel four times faster than the space shuttle; houses would cost 200 bucks. What's the secret behind electronics stunning advances?
How many times have I reviewed these and wondered exactly what's inside there?
Do you mind if I have a look?
AMY KOPPMAN: No, not at all. Go ahead.
DAVID POGUE: I'd like to have a look inside.
AMY KOPPMAN: Yeah, please do.
DAVID POGUE: Where I come from, you want to know how something works, you cut it open. Sony versus saw; here we go.
You guys standing far enough back? Because I don't want anyone to get hurt. What do you think we'll find? Elves? Butterflies?
And now, let's see what really is on the inside of a digital camera. Not much really, and no moving parts at all! This digital camera...
AMY KOPPMAN: This is the brains.
DAVID POGUE: ...runs on a half-inch-wide microchip.
So it seems like this is really the heart of the camera. A lot of it just exists so that I can handle it with my big human hands.
AMY KOPPMAN: Correct, 'cause that's not exactly the most comfortable form factor you want to be using right there.
DAVID POGUE: I know.
Honey, smile. Come on, let me see you smile. Come on.
This tiny wafer contains a highly sophisticated machine. What's it made of?
A computer chip is like a densely packed city: a solid slab of silicon, sprinkled with other elements like boron and arsenic, topped by layers of metals and ceramics. They are laid out like tiny functional neighborhoods.
Over here is memory.
Fifty years ago, you'd have needed a whole building full of vacuum tubes to store just a fraction of what fits in here.
Over here is where data comes in and out of the chip.
Fifty years ago, the fastest computer on Earth could process, maybe, a few hundred punch cards a minute. Today, data goes in and out billions of times faster.
And here is the processor.
Fifty years ago, a computer could add a few thousand of numbers in a second. In the same amount of time, this tiny chip can perform billions of calculations.
Scientists have discovered that the secret to cheap computing power is size. When we find the right materials, and make them small, they change the world.
The race to miniaturize began 500 years ago, with an invention that, in its day, was the first personal computer. I'm talking about the watch.
How did they go from big wall-mounted grandfather clocks to something you could wear on your wrist?
Pierre Gygax (Chief Operating Officer, Ulysse Nardin): The miniaturization, more functions in a smaller space.
DAVID POGUE: Pierre Gygax is a watchmaker, in Switzerland. Some of his watches have more than 400 components.
And how small are some of the parts?
PIERRE GYGAX: There are parts, which are point-zero-zero-six millimeters. So that means a half the thickness of a hair.
DAVID POGUE: Wow. Hundreds of precision metal pieces all driven by a simple mechanism that all clocks have in one form or another: the oscillator, the beating heart of the machine. It's the piece that puts the tick and the tock in time.
PIERRE GYGAX: You know the time is flowing. And it's always difficult to measure something flowing. So, what we do is we, we cut the time in slices. And the oscillator is counting the slices.
DAVID POGUE: The original oscillator was the pendulum, slicing like a knife through time, with each swing counted by the movement of circular gears.
But pendulum clocks work only if they are upright in a fixed position. So, in the Middle Ages, clocks were confined to immovable structures like towers or furniture. But in the 15th century, the invention of the mainspring changed everything.
It was essentially the first battery, a metal coil that could store mechanical energy. As it unwound, the mainspring powered a compact wheel. It was a major breakthrough. Suddenly, gravity and the pendulum were no longer necessary.
The new spring-driven mechanism made it possible to shrink the clock to fit into a hand or a pocket, and the pocket watch was born.
PIERRE GYGAX: This watch is absolutely amazing. It shows the exact position of the sun and the moon, all around the earth, from the top of the North Pole.
DAVID POGUE: Oh, man, that's really cool. And how much does this watch go for?
PIERRE GYGAX: Between $80- and $90,000.
DAVID POGUE: But no need to spend 90 grand to find out what time it is. Nowadays, super accurate watches are disposable, thanks to another great clock revolution, which began in the 1960s.
Out went the spring and mechanical oscillator, replaced by a tiny sliver of solid mineral quartz.
Slice a piece of quartz small enough, send an electric current through it, and it vibrates fast. A quartz-driven clock can accurately chop time into millionths of a second, but the biggest selling point for quartz is that it's cheap.
That's because quartz is actually silicon, commonplace sand, the second most abundant element on Earth.
For the first time, a material replaced a machine, opening the door to a new era of miniaturization. But silicon can do more than just mark time. It's a member of a strange class of elements called semiconductors, found on the table of the elements.
As the name implies, they occupy a middle zone between metals, which conduct electric current, and insulators, like rubber and plastic, which don't.
Think of water flowing through a pipe, an insulator is like a pipe that's frozen: electrons can't get through. Semiconductors are materials that change from free-flowing conductor to a frozen insulator and back again, simply by zapping them with an electric current.
Switches made out of semiconductors are called transistors, and their amazing on-again, off-again switching ability made the computer revolution possible.
But how did they get to be so small?
One great place to look for answers is Intel, a pioneer in squeezing tiny transistors onto computer chips.
I've met a lot of scientists who talk about switches and semiconductors, and somehow they are fulfilling the same function, but, but what is it?
Stephen L. Smith (Vice President, Intel Corporation): What we're trying to build with a semiconductor is a switch. This is one from the, the wall, something you'd use to turn on a light and turn off. And, in fact, when we push the switch up, we give an input and the light is an output.
DAVID POGUE: So, in science fair terms, a switch then, lets electricity go through or stops it.
Stephen Smith: Exactly. Based on the input, we change the flow of electricity.
DAVID POGUE: Electricity on or off. It's the only language computers understand. When the switch is off, the computer reads a zero. When the switch is on, the computer reads a one. String a bunch of switches together and you can create a code. With just eight switches, you can represent any symbol on a keyboard. For a page, you need about 25,000 switches. One-point-four million will get you a second of music. Photos need tens of millions. And videos? We're talking about tens of billions. The more switches, the more power.
The story of the computer revolution is the story of the shrinking switch. Early computers used mechanical relays and vacuum tubes as switches. Building a machine with just a few thousand took up rooms of space. But the silicon transistor changed all that. Because it's a material, not a machine, it's easy to shrink.
STEPHEN SMITH: The exciting part about silicon transistors is we're actually using the atomic properties of the silicon. So rather than actually having to craft something, to build a switch, to build the pieces, to build a spring, I actually, by doing some smart engineering, can get the electrons to flow by using the properties of the atom. And we brought some material to illustrate that. What we have here...
DAVID POGUE: We happen to have a hunk of cheese lying around the lab?
STEPHEN SMITH: A hunk of cheese, a hunk of cheese.
So think of this as the silicon material. I can actually take a slice of that silicon, and I can use the atomic properties of this slice to build those transistors.
DAVID POGUE: Ladies and gentlemen, the Pentium-cheesium-five. Um, I understand that it works really well with the computer mouse. (You can use that.)
All right, so you're saying that one beauty of silicon is that you can cut it in half and it's still silicon?
STEPHEN SMITH: Exactly.
DAVID POGUE: And you can slice it again, smaller and smaller and smaller, but it still does just as good a job at passing along the ones and zeros.
STEPHEN SMITH: Absolutely. And I can use those material properties until I get down to the size of only a few atoms of silicon.
DAVID POGUE: Wow. Which is not something you could do to make mechanical switches smaller, right? Like, if I wanted to make this smaller, you know, I can't just go like this.
STEPHEN SMITH: Wow!
DAVID POGUE: And you can have a smaller one. Clearly, this is not going to be a smaller, good switch.
STEPHEN SMITH: Right.
Uh, but this is silicon. It is a purified element that one mines.
DAVID POGUE: All right, so what does it look like in the computer, then?
STEPHEN SMITH: Well, by the time it gets to the computer, it actually is one of these devices. So, this shiny surface is a piece of refined silicon. It has transistors built into it. We've actually flipped it over so that the transistors are on the other side, and what you see is the back of that piece of silicon.
DAVID POGUE: And this is how many of those little on-off switches?
STEPHEN SMITH: This is almost a billion transistors.
DAVID POGUE: Wow. A billion switches on a one-inch chip.
What's even more astonishing is that one of the founders of Intel saw this coming. In the '60s, Gordon Moore predicted that the size of transistors would shrink by half every two years, each time, doubling the number that could be squeezed onto a single chip.
This idea is known as Moore's Law, and it has proved to be incredibly accurate. But now, 50 years later, Moore's Law may finally be running out of steam. The transistors that power our stuff are about as small as they can get, unless scientists can come up with a new way of packing them ever-more tightly together.
To see one of those possible solutions, I've crossed the country to visit the IBM Research and Development..."kitchen?"
So this is Moore's Law of Italian cooking?
Frances Ross (IBM Research Division): That's right. What we're going to do is explain why it's so important to get the transistors smaller and smaller.
DAVID POGUE: Frances has a pretty appetizing way of visualizing this law and its limitations. Like pepperoni slices, the transistors on a silicon chip are flat.
Okay, so here's our...
Frances Ross: Silicon wafer.
DAVID POGUE: ...silicon wafer.
Frances Ross: Now, these are the old-fashioned transistors. They're much larger, and you can see that you can't put that many onto each wafer.
DAVID POGUE: So this would be a 1960 iPod?
Frances Ross: I think so, yes. This would be a '60s type of thing.
So, let's take off these old transistors and replace them with some new transistors.
DAVID POGUE: Oh, these are much smaller!
Frances Ross: Yes, these new transistors are much smaller.
DAVID POGUE: Technology has marched on.
Frances Ross: That's right. It's Moore's Law, in action.
DAVID POGUE: So, in other words, all we have to do is make the transistors smaller every year, forever, and our gadgets will always be more powerful and more compact.
Frances Ross: That would be wonderful, but we can't make our pepperoni slices much smaller than this. And these transistors are now packed together about as close as we can get them.
DAVID POGUE: The pizza party can't go on forever. There's a limit to how small you can shrink the transistors. If you reduce the surface area of a transistor too much and place it too close to its neighbor, electricity starts to leak, causing a short circuit. Not good.
Frances Ross: We've run out of area, so there's only one way to go, and that's upwards.
DAVID POGUE: Slim Jims?
Frances Ross: That's right. This is a vertical transistor. Instead of having flatter, smaller transistors we go in the other direction.
DAVID POGUE: Excuse me, vertical transistors?
Frances Ross: Vertical transistors.
DAVID POGUE: With little toothpicks on the bottom?
Frances Ross: That's just for demonstration purposes.
DAVID POGUE: Oh, okay.
By building vertical transistors, called nanowires, Frances can increase surface area, without bringing the transistors closer together, so no short circuit.
Ingenious. So this is what you're doing at IBM? You are making these?
FRANCES ROSS: That's right. They're called nanowires, and the real thing is about a million times smaller than this.
DAVID POGUE: A million times smaller?
FRANCES ROSS: That's right.
DAVID POGUE: Well, that would be hard to see!
They're hard to see. But this is not a nanowire. This is a silicon sliver Francis uses as a surface to grow them.
FRANCES ROSS: We get tens of millions of wires on each of these specimens.
DAVID POGUE: Come on!
FRANCES ROSS: Yes.
DAVID POGUE: Now you're hurting my brain.
FRANCES ROSS: Oh.
DAVID POGUE: She carefully loads the wafer into a molybdenum clip and slides it into a custom-built oven, where she'll bake it at 1,100 degrees Fahrenheit.
You know, I was just thinking, Frances. I don't think you have enough aluminum foil on this oven.
FRANCES ROSS: Yes, it's the question that everyone asks. It holds the heat better.
DAVID POGUE: Aluminum foil?
FRANCES ROSS: That's what, that's what we use.
DAVID POGUE: Isn't that a little low tech?
FRANCES ROSS: That's right; whatever works.
DAVID POGUE: Oh, my gosh. So those little spires...?
FRANCES ROSS: Those are the nanowires.
DAVID POGUE: So, you bake those up?
FRANCES ROSS: Just grew these, yes.
DAVID POGUE: We can see them, because this oven doubles as an electron microscope.
All right, so these are them, huh?
FRANCES ROSS: This is 30,000 times magnified.
DAVID POGUE: Thirty-thousand times?
FRANCES ROSS: That's right. So here's the column of silicon that's the nanowire, and here's the gold droplet, on the end, that actually makes it grow.
DAVID POGUE: It's weird. It looks like matchsticks or weird mushrooms.
FRANCES ROSS: They do...mushrooms, that's right. They look to me like mushrooms.
DAVID POGUE: That's amazing.
FRANCES ROSS: So we're trying different catalysts, different recipes, but this here is the future of transistors
DAVID POGUE: Wow.
While scientists like Frances try to find ways to push silicon to its limit, others are pinning their hopes on a new material that lets electrons flow a thousand times faster than they can in silicon. And it's called "graphene."
Pablo Jarillo-Herrero (Physicist, Massachusetts Institute of Technology): When graphene happened, I just couldn't stop myself from going into it. It was so beautiful, I just couldn't stop. I immediately jumped onto it.
DAVID POGUE: This is Dr. Pablo Jarillo-Herrero, a professor at M.I.T. and graphene guru.
PABLO JARILLO-HERRERO: So, here I have graphene. Graphene is the thinnest material that exists. This is just one-atom-thick, okay, sheet of graphene and you can see that it is perfectly visible. So, it's part of the magic of graphene. You can just see it, even with your eyes.
DAVID POGUE: You heard that right. This grey square of graphene is just a single atom thick. Although graphene was discovered only recently, it's been hiding in plain sight for ages, in a material you probably have on your desk: graphite, also known as pencil lead.
PABLO JARILLO-HERRERO: You can write with a pencil because graphite is a layered material. And as you write, you are leaving traces of these layers on your piece of paper. So graphene is really just one sheet of this graphite material, a one-atom-thick sheet.
DAVID POGUE: That makes graphene an ideal conductor. At only one atom thick, there's nothing to restrict free electrons, which flow across the surface of the material, like water across flat ice.
PABLO JARILLO-HERRERO: Graphene is a very special conductor...is the best conductor, and we're now studying those properties and learning how fantastic this material is.
DAVID POGUE: And scientists have also figured out how to make transistors out of graphene, giving it the ability to speak the language that electronics and computers understand.
PABLO JARILLO-HERRERO: So, I'm excited. It's beautiful. Here you have a material that will enable high-speed, ultra-high-speed electronics at very low power.
DAVID POGUE: But it gets even better. Turns out, the only tool you need to make graphene is a piece of tape.
PABLO JARILLO-HERRERO: It is so simple, any high school student can indeed make one atom-thick devices with this. It's really amazing. That scotch tape is going to be folded into two, and then, when we separate that tape, this graphite naturally exfoliates in two pieces. Then we're going to fold it again. It will split into four pieces. Then eight. Do it again and again, making the piece of graphite thinner and thinner and thinner. Basically until we cover the entire tape with graphite.
We're then going to take a silicon chip, deposit it on top of the tape. And what we're hoping is that the graphite pieces which are on the tape are going to get in intimate contact with the silicon. So when you remove the chip and you look then with an optical microscope, you can see the one-atom-thick material. And that's graphene.
DAVID POGUE: Graphene promises to make the impossible possible, letting electrons move across its surface at virtually the speed of light and generating almost no heat. In fact, graphene is such a revolutionary material that in 2010, a mere six years after its discovery, the two Russian scientists who first made it received the Nobel Prize in Physics.
The computer chips of tomorrow could be a quantum leap forward: computers with nearly limitless processing power; every book ever written, stored on a tiny chip; a highway system so smart it could control millions of cars without a single accident. And it's not just about our gadgets, it's about us.
While the electronics story continues to unfold in amazing ways, the story is beginning all over again with a materials revolution in medicine.
It's not a new idea. Remember this?
Audio Clip (Fantastic Voyage, Film Clip) Phase One calls for miniaturizing a submarine and injecting it into the carotid artery.
DAVID POGUE: Fantastic Voyage, it was the sci-fi smash of 1966.
Audio Clip (Fantastic Voyage): Phase One, Phase One.
DAVID POGUE: Scientists shrink a team of doctors and send them into a sick man's body, on a mission to cure him.
Audio Clip (Fantastic Voyage): Stand by for injection. All stations stand by. Tracking post. Inject.
DAVID POGUE: Today, as our devices get smaller and smaller, Fantastic Voyage is beginning to look like prophecy, the kind of thing that can change lives.
DANIEL MISHKIN: Hi, Courtney.
Courtney DeSisto (Patient): Hi, Dr. Mishkin. How are you?
DANIEL MISHKIN: Good and yourself?
COURTNEY DESISTO: I am good. Thank you.
DAVID POGUE: Today, Courtney will be taking a pill, but it's not just any pill. It's a miniaturized camera.
DANIEL MISHKIN: This capsule is a miniaturized camera. Every time it blinks it's actually taking a picture. It's acquiring images at a rate of two-frames per second.
COURTNEY DESISTO: Okay.
DANIEL MISHKIN: And what I'm going to get you to do is actually to swallow the capsule.
COURTNEY DESISTO: Okay.
DANIEL MISHKIN: And as the capsule goes through the G.I. tract it's going to be taking pictures of what's going on inside.
COURTNEY DESISTO: Wow.
DAVID POGUE: It's called the PillCam, and it travels through the body just like a piece of food, taking 55,000 pictures, over the course of eight hours.
DANIEL MISHKIN: Why don't we actually put it inside your hand?
DAVID POGUE: Pictures that can provide a diagnosis that once would have required surgery.
DANIEL MISHKIN: All right, so as you move it around, we can actually see the folds of your hands, with excellent magnification. So once we actually go ahead and ingest the capsule, it's going to give us that same magnification of what's going on inside.
COURTNEY DESISTO: Wow, that's really cool.
DANIEL MISHKIN: It actually has a wireless transmitter, that's going to transmit the images to the data recorder that you'll be wearing over the course of the day, and I'm going to download the images and be able to look at them and analyze exactly what's going on.
COURTNEY DESISTO: Okay.
DAVID POGUE: The PillCam is made of an inert plastic that doesn't create a toxic response in the body. Inside, is a mini-catalog of the electronics industry: a tiny video camera and flash, a radio transmitter, a battery, and, of course, a computer chip to drive it all. Twenty-five years ago, all of those components would have taken up a cubic yard of space. Today, it all fits inside a one-inch capsule that weighs only a fraction of an ounce.
DANIEL MISHKIN: So let's go ahead and ingest it.
COURTNEY DESISTO: Okay.
DANIEL MISHKIN: Ready? Into your mouth. I'll give you a glass of water. Now I see your teeth. And, go ahead, down the hatch. Okay, great. So that's it. That's the hardest part.
COURTNEY DESISTO: Okay.
DANIEL MISHKIN: Just remember, over the next two hours, do not drink anything.
COURTNEY DESISTO: Okay.
DAVID POGUE: As the PillCam moves through Courtney's digestive tract, it records what it sees, eventually giving Dr. Mishkin a front-row seat as he looks for abnormalities.
DANIEL MISHKIN: Right now, we're looking at the small intestine. It's able to see 360 degrees, such that it's like looking down a gun barrel.
The capsule is great at acquiring images, but I'm hoping that as the next generations of the, this capsule develop that it's not only be able to take pictures, but it will eventually be able to biopsy—sample the tissue in that area—or even deliver a treatment, such as placing a clip on a bleeding site, or even deliver medications.
DAVID POGUE: The PillCam is the pocket watch of today, a super-miniaturized machine that liberates the patient. It took centuries to make the leap from mechanical watches to computers, but in the world of microscopic medicine, the story of smaller is unfolding on a vastly accelerated timeframe.
In fact, scientists are on the verge of realizing a 21st century version of the Fantastic Voyage story. They are developing microscopically small robots that travel into the body's deepest reaches to diagnose, treat, and even destroy deadly illnesses.
This is your lab?
BRAD NELSON: This is my lab.
DAVID POGUE: This is where you build your robots?
BRAD NELSON: This is where we build the robots.
DAVID POGUE: This is Brad Nelson. He's created a robot that could help cure blindness.
Oh, nice, it's incredibly lifelike.
BRAD NELSON: Yeah, that's a mannequin.
DAVID POGUE: Oh, sorry.
BRAD NELSON: These are the ones we build.
DAVID POGUE: What? That's a robot?
BRAD NELSON: That's a robot.
DAVID POGUE: Looks like a splinter.
BRAD NELSON: Well, this is a microrobot. We use them to help perform surgeries on the eye or inside of the eye.
DAVID POGUE: The device is only a hundredth of an inch wide, small enough to fit into the needle of a syringe, like the tiny sub in Fantastic Voyage. But the similarity ends there.
This unmanned device is designed to treat a type of blindness caused by blocked blood vessels in the retina, the tissue where images are formed. The robot delivers an extremely small dose of medicine to restore blood flow and vision.
That makes me think that this little tiny thing has batteries and little propellers and some kind of knowledge to know where to go in the eye. I have a hard time believing that.
BRAD NELSON: That's right. The way we energize is we use externally generated electromagnetic fields. So, basically, it's a magnet.
DAVID POGUE: To make the device small enough, Brad had to abandon the idea that robots have to be mechanical. Instead, he focused on finding a material that would let him eliminate bulky moving parts.
He chose two elements, samarium and cobalt. Combined, they form a material highly sensitive to magnetic fields, which means that Brad can direct the movement of the robot without touching it.
Once again, a material replaces a machine and the device gets smaller.
BRAD NELSON: So then, besides just the robot, what we also have is this system here, of electromagnets, and so what each of these copper coils do, is they generate magnetic fields.
DAVID POGUE: Oh, man. Yeah, you got a bunch of them in every direction.
BRAD NELSON: So we have eight of these here. That's why we call this the OctoMag.
DAVID POGUE: OctoMag?
BRAD NELSON: That's right.
DAVID POGUE: The OctoMag. By adjusting the strength of these eight electromagnets, the surgeon can move the microrobot any direction along the x, y and z-axes, pushing or pulling it through the eye. But landing it on the tiny section of retina used for seeing in sharp detail takes lots of practice.
And you've been practicing with dummy eyeballs, so far?
BRAD NELSON: We use that, but we also use animal eyes as well. We get pigs eyes from our local butcher.
DAVID POGUE: You buy eyeballs from the butcher, from cadavers?
BRAD NELSON: That's right, Christos, one of my PH.D. students...Christos goes in the morning, early in the morning, to the butcher and asks for eyeballs.
DAVID POGUE: Christos. How are you?
Christos Bergeles (Student, Institute of Robotics, ETH Zurich): Fine.
DAVID POGUE: Oh, my. These are the pig eyes?
Christos Bergeles: Exactly. Twenty pig eyes fresh from the butcher, ready to be prepared for experiments.
DAVID POGUE: You must be a big hit around Halloween.
And what about this guy, what's this all about?
Christos Bergeles: Ah, we call him Mr. Pig Eye Guy.
DAVID POGUE: Mr. Pig Eye Guy?
Christos Bergeles: Well, you have to give them a name. Then we put the eyes in this hole here.
DAVID POGUE: Once the eyeball is secured inside the socket, we insert a light probe.
Christos Bergeles: That's the light there.
DAVID POGUE: Oh, okay. I can actually see the light in there, very cool.
This tiny L.E.D. lets us see what we're doing when we drive the robot.
So, this is the robot right here?
Christos Bergeles: Mm hmm.
DAVID POGUE: Okay. And this isn't fake? We're really seeing this live from the microscope, right now, right?
Christos Bergeles: Yes. Right now. You can move this guy around.
DAVID POGUE: Okay. Sure enough, I push right, he goes right; left, he goes left. Let's see when I go down over here.
Christos Bergeles: And then you can pull up and you can see it.
DAVID POGUE: Oh, it's getting bigger.
Christos Bergeles: Exactly. This means that it's moving higher, away from the retina.
DAVID POGUE: Closer to the surface of, oh man! I think I just banged the top of the cornea or whatever you call it.
Christos Bergeles: Exactly. So that's the very top, and this is where the liquid ends. So you see this, this effect.
DAVID POGUE: Now let me push down.
Christos Bergeles: When you push down, it starts slowly going into the retina.
DAVID POGUE: It's sinking, sinking! Wow, that's really cool.
Christos Bergeles: So, now you've reached the bottom; you're touching the retina.
DAVID POGUE: Yeah, it's quite responsive. I can go right up here.
Eventually, Brad hopes that there will be a commercial version of his device installed in doctors' offices. The magnets will be arranged in a housing that surrounds the patient's head while the doctor peers through a microscope to guide the drug-filled robot.
Brad's initial success combining materials and magnetic fields to make tiny devices has encouraged him to be even more ambitious. His goal is to build a robot that can swim through blood vessels.
But, along the way, he's discovered that the smaller you go, the stranger the world becomes.
Oh, dear, looks like the grad students have left their things out again.
And the harder it is to get around.
BRAD NELSON: So what we've seen, so far, are the microrobots for the eye. We're interested in going even smaller and trying to make smaller robots. So I set up an experiment here to, kind of, show you what some of the problems are when we try to make small things swim, and why it's so different from how big things, like fish or the toys like this, swim in water.
DAVID POGUE: Okay.
BRAD NELSON: So what we've got here are two tanks. One is just regular water, out of the tap. This other one is glycerin. It's much thicker, like oil or corn syrup, something thick like that. So let's look at how something like this toy goldfish is going to swim in water.
DAVID POGUE: Wind her up?
BRAD NELSON: Wind her up. There you go.
DAVID POGUE: Okay, the tail goes back and forth.
BRAD NELSON: Just the way you expect.
DAVID POGUE: I'd say that swimming is fairly effective.
BRAD NELSON: Okay, he's got a twin over there. Let's see how he does in the glycerin.
DAVID POGUE: And this one is going to go in the goop. He wags his tail and doesn't move at all.
BRAD NELSON: This helps us illustrate how water feels when you get small. If you made yourself 10,000 times smaller and you jumped in this pool of water, it would actually feel more like you were swimming in glycerin here, or goopy stuff, like honey, or something thick like that.
DAVID POGUE: Right. A swimmer the size of a bacterium would never be able to get around using flippers or the breaststroke, because, at that size, the friction from the water molecules becomes a major drag.
For a long time, scientists couldn't figure out how bacteria were able to swim, but, eventually, they discovered the secret. The tail, or flagellum, seems to move back and forth, but viewed from another angle, it's clear that it moves in a totally different way.
Corkscrew tail?
BRAD NELSON: A corkscrew tail.
DAVID POGUE: Brad and his team have developed corkscrew robots that mimic bacteria.
BRAD NELSON: Bacteria like E. coli and salmonella have developed these flagella that twist like...
DAVID POGUE: This part is the flagella?
BRAD NELSON: These flagella twists through the liquid, just like a corkscrew going into a bottle of wine. So instead of propelling itself or using its inertia, it's actually, kind of, cutting through the fluid.
DAVID POGUE: It's almost pulling itself, instead of pushing itself.
BRAD NELSON: It's pulling itself, in a sense, like a screw going into wood or something like that. It's a completely different material interaction.
DAVID POGUE: Very cool.
They use the same samarium-cobalt material and magnetic fields to set them in motion.
So, it's literally, like, drilling its way through that?
BRAD NELSON: Drilling its way up.
DAVID POGUE: Wow.
This is the biggest one. The smallest one is only 30 microns long. That's 30 millionths of a meter, about a third the width of a human hair.
BRAD NELSON: So, now, just in the last few years, we've been able to build small things, of a similar size and shape to real bacteria, that swim just like they do, potentially, deep inside a person's body.
DAVID POGUE: The magnet-driven robots in Brad's lab: the eye bot, the flagella bot, and even a soccer-playing robot that his students created, are each no bigger than a dust speck.
Brad's learned to modify his robots to overcome the physical obstacles in the microscopic realm, but he's only scratched the surface of the strange properties in that infinitesimal world.
Chad Mirkin (Chemist, Northwestern University): An atom is actually a fraction of a nanometer.
DAVID POGUE: Chad Mirkin is an explorer and pioneer in this weird realm: the nano world.
You keep saying you're building things on the nanometer scale. I don't even know what a nanometer is. So, this is a meter, this is a centimeter, this is a millimeter, is this a nanometer? What's a nanometer?
CHAD MIRKIN: Let me try to illustrate it for you. If we shrink you by a factor of two, you're about the size of a small child. We continue to shrink you by a factor of two, so, four more times, you're about the size of a golf ball. We go to ten, you're now the size of an ant. We keep going another seven times, and now you're about the diameter of a human hair. That's roughly what we can see with the naked eye.
DAVID POGUE: Even after so much shrinking, I'm not even close to the nano scale. Cut me in half five more times and I'm the size of a red blood cell. Five more times, I'm a virus. Seven more times, and I'm finally one nanometer in size, one billionth of a meter. That's less than half than the width of D.N.A.
It seems unimaginable that we might harness materials this small, but, in fact, it's not all that new.
CHAD MIRKIN: People have been using nanotechnology almost unknowingly for centuries. For example, back in the Middle Ages, when stained glass windows were made, they were using tiny little particles to get the beautiful colors. You simply need to go to Canterbury Cathedral and you can see the effects of nanotechnology in the beautiful glass windows.
DAVID POGUE: Canterbury Cathedral: some of the stained glass in here is nearly a thousand years old. With so much history under one roof, it's no surprise that the Cathedral needs a fulltime staff of glass preservationists.
So this is the paint station. This is like my local Home Depot, with different swatches, right?
Leonie Seliger (Head of Cathedral Studios): It's a little bit like that, yes.
DAVID POGUE: This is Leonie Seliger, the head restorer at the Cathedral.
I've come in search of ancient nanotech secrets.
LEONIE SELIGER: Um, what we're looking at here are stains. The stain gets fired. It's like pottery glaze; it's fused onto the surface of the glass.
DAVID POGUE: Okay.
LEONIE SELIGER: The trouble is that you don't just paint a yellow color on glass and you know how deep and how rich it is. You have to use a chemical process. So to make yellow, you mix silver with clay. Silver, under heat, actually produces a yellow glass.
DAVID POGUE: Wow!
She's using silver chloride, which in its natural form looks like small, silvery crystals. Leonie mixes a tiny amount with red clay.
LEONIE SELIGER: It's a silver salt that's mixed with this clay. You can't see it, what you see is the clay.
DAVID POGUE: Okay, okay.
LEONIE SELIGER: You would then paint that on. I've made a little series here, where I've now applied this clay, and after it's fired, if I then wipe that off...presto.
DAVID POGUE: Oh, look at that. From silver clay comes a golden color. There's some kind of voodoo chemistry going on there for sure.
LEONIE SELIGER: That's the mystery.
DAVID POGUE: Somehow this is nanotechnology at work. In the heating process, the silver crystals break down into tiny nanoparticles and turn yellow.
LEONIE SELIGER: But it only works on this glass.
DAVID POGUE: And that's not all. Leonie assures me there are several metals used in stained glass where nanotech creates surprising color results.
LEONIE SELIGER: Copper will give you...
DAVID POGUE: Brown?
LEONIE SELIGER: Or red.
DAVID POGUE: Red?
LEONIE SELIGER: Or green.
DAVID POGUE: That's just bizarre.
LEONIE SELIGER: Gold gives you beautiful rich pink glass, even rich ruby glass.
DAVID POGUE: And why is that? Why is something we think of as gold, why does it come out red?
LEONIE SELIGER: Ask a chemist.
DAVID POGUE: It's magic; it's religion.
LEONIE SELIGER: It is a bit. In the Middle Ages, of course, it was always a closely guarded secret on what makes what color at which temperature.
DAVID POGUE: When artists first learned how to change the colors of metals, it must have seemed like alchemy, but, in fact, it had something to do with changes at the smallest possible scale.
I want to get to the bottom of that mystery.
So this is your inventory here?
Mike Tuffey (Owner, English Antique Glass): This is our stock.
DAVID POGUE: So, I'm digging a little deeper at the English Antique Glass company, which makes the colored glass used to repair Canterbury's windows.
MIKE TUFFEY: There's cobalt blues.
DAVID POGUE: Wow. These sheets are crafted by hand, still using the same techniques that were developed in the 12th century.
How many establishments are there?
MIKE TUFFEY: Making this?
DAVID POGUE: Yeah.
MIKE TUFFEY: One. We're the only makers of flat glass, traditional methods, in the U.K. and Ireland.
DAVID POGUE: Wow.
This is Mike Tuffey, the head of production here. He and his team of glassblowers are able to achieve the same rich colors found in the cathedral, but they start with clear glass, which is made from a mix of sand, limestone, sodium carbonate and other minerals.
Oh, my gosh, that's it?
MIKE TUFFEY: That's it.
DAVID POGUE: That's the next great cathedral window right here?
MIKE TUFFEY: That's the next great cathedral window right there.
DAVID POGUE: It looks like kitty litter; it doesn't even look like glass.
MIKE TUFFEY: We just put that in the furnace. This is a rod we use for the different colors.
DAVID POGUE: The rods contain concentrated amounts of the metals that create color when added to the clear glass and fired at 1,200 degrees Fahrenheit.
So what does it look like when it comes out? Is it a sheet? Is it a blob?
MIKE TUFFEY: It's a blob.
DAVID POGUE: The journey from molten blob to colored glass is an intricate process of shaping, blowing and re-firing, resulting in a glass cylinder called a muff.
It's this heating and cooling process that creates the final color, thanks to the action of the metal nanoparticles.
You slit it, and then you have a flattening machine that uncurls it?
MIKE TUFFEY: That's right, that's it.
That's a gold, pink muff.
DAVID POGUE: There's gold dust in there?
MIKE TUFFEY: Yes.
DAVID POGUE: But it doesn't look gold, it looks pink.
MIKE TUFFEY: No, gold gives you pink.
DAVID POGUE: Gold gives you pink?
MIKE TUFFEY: Gold gives you pink.
DAVID POGUE: Why would that be?
MIKE TUFFEY: Ah, you would need to talk to a chemist on that one.
DAVID POGUE: I plan to do that.
Same answer. I'm 0-for-2 with the stained glass people.
So I went to Canterbury Cathedral, and I actually spoke to the guy who makes the glass, and the lady who does the repairs on those windows, and they said sure enough, they add gold to the glass to make it red. It doesn't make any sense, and you know what they told me? "We have no clue." But you're the scientis, man, you should, you should be able to explain why gold makes red.
CHAD MIRKIN: Well, it turns out that if you can control the size of a gold particle, if you can shrink it to this nanometer-length scale, you have completely different optical properties. Gold is no longer gold when taken to the 13-nanometer size, it's ruby red in color.
DAVID POGUE: When light rays hit a colored material, some colors are absorbed and some are reflected. That's why roses are red and violets are blue. Many metals, like gold and silver, reflect most of the colors in visible light, which is why they can be polished to shine like mirrors. But when a particle of gold is made very small, below 100 nanometers, 100 billionths of a meter, the particle begins to absorb shorter wavelengths of light, toward the blue end of the spectrum. The smaller the particle, the more blue is absorbed and the redder it appears.
But it gets even stranger, because not only size matters, shape does too.
Each of these vials contains water with silver nanoparticles dissolved in it. The only difference between them is the shape of the particles. In this test, silver rods give you yellow; silver triangles, green; silver prisms give you blue.
If metals behaved like this in our big world, then just changing the size or the shape of your car would alter its color.
Chad sees tremendous potential in this weird nano-phenomenon.
CHAD MIRKIN: With almost an infinite number of possibilities, you no longer have to take what nature gives you. You can adjust color simply by becoming a nano-architect.
DAVID POGUE: Scientists call this strange property of small materials "structural color." The living world figured this out millions of years ago. Structural color on the nano-scale creates the iridescent pigments in butterfly wings, beetle shells and peacock feathers.
Well, why do we care? I mean cool, little tiny gold particles are really red. I mean how does that help mankind?
CHAD MIRKIN: Well, the reason we care is that once you discover new properties, those new properties almost always lead to new applications.
DAVID POGUE: There are already a number of medical applications. Chad Mirkin has developed a technology that harnesses the unique properties of gold and silver nanoparticles to test the genetic variations in patients.
Sequencing D.N.A. is expensive and time-consuming, but Chad's revolutionary test takes less than two hours.
I offered to bleed for science, to see how it works.
Roy Pritchard (Technician): And your name is?
DAVID POGUE: David.
Roy Pritchard: Okay, so we're doing a low blood draw today. You can relax.
DAVID POGUE: Is this the extraction bench?
Tram Truong (Technician): Yes, this is.
DAVID POGUE: First, a technician extracts pure D.N.A. from my blood sample.
This is my DNA?
Chris Riley-Portugues (Technician): This is your D.N.A.
DAVID POGUE: He then loads it into a small, disposable cartridge and inserts it into the machine for testing.
This test can actually read the letters of my D.N.A. And, using gold nanoparticles, it flags variations that might make me unusually sensitive to particular drugs, or even mutations that signal heightened risk for disease.
Less than two hours after drawing my blood, the results are in. It turns out that the test has some interesting news for me about my sensitivity to a blood-thinning drug called warfarin, or Coumadin. It's commonly prescribed to stroke and cardiac patients.
It's a potentially lifesaving drug, but if the dosage is wrong, it can cause fatal bleeding.
So what's my warfarin dosage?
Jean Lopategui (Cedars-Sinai Medical Center, Molecular Pathology): What they call a double hit, I'm sorry to say.
DAVID POGUE: A double hit?
JEAN LOPATEGUI: You're a double hit, so you have two genes that are mutated, and therefore you're very sensitive to warfarin. Your calculated dose is 2.7 milligrams.
DAVID POGUE: See, I knew it! My mother always said it would be like 2.6 or 2.5, but I always said, "No, Mom, mine's 2.7."
JEAN LOPATEGUI: You should always listen to your mom, David.
DAVID POGUE: The nanosphere test gave me some crucial information...
Chris Riley-Portugues: This is the mutation.
DAVID POGUE: Ahh.
...quickly enough to save my life, if I'd been ill.
It's a diagnostic tool. The next goal is to fight illness in the body, at the same tiny scale.
SAM WICKLINE: Okay, so, when we inject this, it will go in the bloodstream and find the cancer. Like a rocket-guided system.
DAVID POGUE: Sam Wickline has invented a nano-device that's smaller than a virus. Engineered atom by atom, his hunter-killer robots are designed to travel by the billions in the bloodstream. They're pre-programmed, by a doctor, to seek out specific types of cancer cells and then destroy them. With none of the side effects associated with current drug therapies. It's the ultimate Fantastic Voyage dream. And it's borrowing a page from these little guys.
Wow.
TED JANSEN (Beekeeper): See, here, now.
DAVID POGUE: Whoa.
Ted Jansen: This is full of honey. See? There's probably 35 pounds of honey in there.
DAVID POGUE: Oh, my gosh.
TED JANSEN: In fact, I'll take it out and show you.
DAVID POGUE: He's brought me to meet beekeeper Ted Jansen, to get a close-up look at the inspiration behind the science.
TED JANSEN: Now, see? Here's one that's already stung me, see?
DAVID POGUE: What, what? But, aren't you going to say "ow" or something? Oh, my gosh.
TED JANSEN: No, that...see that's the little venom sac that they leave. But it's not the stinger that hurts you, it's the venom going in you.
DAVID POGUE: A bee sting may seem like a minor irritation, but actually the venom is extremely toxic to cells.
TED JANSEN: Immediately, when you get it out of there the, the pain stops.
DAVID POGUE: So why would it have been bad for you to just pluck it out?
TED JANSEN: Because you squeeze the sack and squeeze all the venom in.
DAVID POGUE: Oh, I see, I see.
So Sam, you're not here to see how they make honey?
SAM WICKLINE: No. We like the bees because they make a toxin. The toxin is melittin, and we use it to, specifically, to treat cancers.
DAVID POGUE: Bee venom is a cancer drug?
SAM WICKLINE: Yes, bee venom is an excellent cancer drug. It's been known for quite some time, but the problem is how to deliver it.
DAVID POGUE: Melittin is not being used yet to fight cancer, because it will destroy any cell it bumps into, including healthy ones. But, realizing its potential, Sam set out to engineer a nano-scale robot to carry the toxin safely through the body and release it only when it finds its target.
Not surprisingly, he calls his invention nanobees.
Each nanobee has three parts.
SAM WICKLINE: So, what we've got here is a model.
DAVID POGUE: Sam explains, with a simple demonstration, how they fit together.
SAM WICKLINE: This basically is a sphere of carbon and fluorine atoms that forms the carrier for that melittin.
DAVID POGUE: The center of the nanobee is a nanoparticle constructed out of several thousand of carbon and fluorine atoms arranged in a spherical cluster less than 300 nanometers in diameter.
SAM WICKLINE: And then we have a coating on that, which is a fatty kind of a coating. And this fatty coating allows us to insert the melittin toxin into this particle here.
DAVID POGUE: They would be all over this thing.
SAM WICKLINE: Exactly.
DAVID POGUE: This outer layer holds the deadly bee poison in place. It's like a holster that keeps a gun safe, until it's drawn.
Every tumor cell has a distinct chemical makeup. The outer layer of the nanobee is programmed to selectively lock onto only those cells that need to be destroyed.
SAM WICKLINE: So, if you take this balloon as a cancer cell. This nanoparticle will come up next to the cancer cell and basically merge with it. The coating will come off, and the melittin itself forms a hole in the cancer cell and pops it.
DAVID POGUE: Like its namesake, a nanobee can only sting once. So swarms of them are required for any treatment. Sam's lab has decoded the chemical makeup of the bee venom, so he can manufacture it in large quantities for his nanobee swarms.
I say goodbye to our beekeeping hosts, in order to pay a visit to Sam's lab at Washington University, to see how the nanobees work in the body.
SAM WICKLINE: So here we are in the magnetic resonance imaging suite. I'm going to show you what it's like to be a patient, as if we were looking for nanobees inside of you.
DAVID POGUE: And may I just say what a boost this gives my dignity to wear a sundress and slide into a giant bagel.
SAM WICKLINE: Well, let's go toast you.
DAVID POGUE: An M.R.I. uses strong magnetic fields and radio waves to detect certain molecules in the body. This machine has been tuned to detect fluorine, which are part of the nanobee molecules but are otherwise very rare in the body.
SAM WICKLINE: Okay, you having fun there yet? We're rolling.
DAVID POGUE: I think I remember this ride. I think I did it at Six Flags.
If Sam had actually injected nanobees into me, and if I had the cancer that they had been trained to fight, they would travel in my bloodstream and stick to any target tissue they encounter. The images that the technicians see would show bright yellow areas where the individual nanobees were finding and killing the diseased cells.
And best of all, there would be no toxic side effects, as with traditional chemotherapy.
So how far away are we from using nanobees in everyday human cancer patients?
SAM WICKLINE: Well, now this material is being tested, uh, in what's called pre-clinical stage. And we hope that, within about a year or so, that we'll have approval to test this in humans.
DAVID POGUE: Well, thanks, Sam. I appreciate this experiment. So, typically, how long would you leave the patient, lying in this tube? Guys? Guys? Hello? Hello?
Like the nanoscale transistors that came before them, nanobees get power from their tiny size. But nanobees and other emerging nano technologies go even further.
SAM WICKLINE: So this is where melittin is made.
DAVID POGUE: Nanobees are part of a new breed of small materials that self-assemble; no need for big complicated machinery to make nanobees. Put the right ingredients together, in the right conditions, and they make themselves.
This revolution in medicine, working at the tiniest of scales, would never have been possible without the revolution in electronics that preceded it. Both are transforming our world and our lives at an astonishing speed.
As we see the power in this new ability to design and build, atom by atom, there may be no more important goal in the science of materials than mastering the art of making stuff smaller.
IMAGE: (David Pogue) ©WGBH/Mark Ostow, ©iStockphoto.com/Luca di FilippoBroadcast Credits
Making Stuff: Smaller
- Host
- David Pogue
- Written, Produced and Directed by
- Chris Schmidt
- Executive Producers
- For Powderhouse Productions
- Joel Olicker
Tug Yourgrau - Co-Producer
- Alexandra McHale
- Field Producer
- Doug Gordon
- Story Producer
- Andy Howard
- Edited by
- Brian Funck
- Additional Editing by
- Kristine Gaffney
- Director of Photography
- Gary Henoch
- Additional Photography
- Tim Cragg
Ken Nilsson
Jesse Phinney - Sound Recordists
- Mark Arees
Steve Bores
Govanni Di Simone
Dan Gleich
Mike Italiano
Mike Keenen
Michael Kranicke
Adam Prescod
Chuck Stanton
James Thompson
Scott Wildfong - Grips and Gaffers
- Ari Manin
Phil Contursi
George Zorich - Production Assistant
- Daniel V. Parsons
- Music
- Lunch Special Music
- Animation
- Edgeworx, LLC
- Additional Animation
- Alan Waldo
Jenny Olson - Production Manager
- Diane Knox
- Post Production Supervisors
- Kevin Young
Michael Fallon - Assistant Editors
- Eric P. Gulliver
Peter Hyzak
Jim Fetela
Bryce Patingre - Online Editor / Colorist
- Julie Kahn
- Audio Mix
- Heart Punch Studio, Inc
- Research
- Sonia Weinhaus
Jessie Lynn Ward - Interns
- Katie Duffy
Nicole Jaques
Travis Kelley
Michael Ryan
Zach Vitale - For Powderhouse Productions
- Post Production Manager
- Melissa Walter Richards
- Senior Production Coordinator
- Carlin Corrigan
- VP of Production
- Daniel Miller
- Senior VP of Production and Post
- Robert Kirwan
- Senior VP of Sales and Development
- Seanbaker Carter
- Archival Material
- AP Archive
Getty Images
Footage Provided by Thought Equity Motion
iStockphoto
Museum of Science Strategic Projects Group
Nathan Baker
Sunjoo Lee
Periodictable.com - Special Thanks
- Producers gratefully acknowledge the cooperation of the Materials Research Society.
- Richard A. Souza
Amy Moll
Kristin Bennett
Jerry Floro
Megan Frary
Kevin Jones
Tommie Kelley
Alex King
Aditi Risbud
Stephen Streiffer
Rick Vinci
Sandra DeVincent Wolf - Special Thanks
- Mimotec
Ulysse Nardin
Professor Stephen Sass, Cornell University - NOVA Series Graphics
- yU + co.
- NOVA Theme Music
- Walter Werzowa
John Luker
Musikvergnuegen, Inc. - Additional NOVA Theme Music
- Ray Loring
Rob Morsberger - Post Production Online Editor
- Michael H. Amundson
- Closed Captioning
- The Caption Center
- Publicity
- Eileen Campion
Victoria Louie
Karen Laverty - Marketing
- Steve Sears
- Researcher
- Kate Becker
- NOVA Administrator
- Kristen Sommerhalter
- Production Coordinator
- Linda Callahan
- Paralegal
- Sarah Erlandson
- Talent Relations
- Scott Kardel, Esq.
Janice Flood - Legal Counsel
- Susan Rosen
- Post Production Assistant
- Darcy Forlenza
- Associate Producer Post Production
- Patrick Carey
- Post Production Supervisor
- Regina O'Toole
- Post Production Editor
- Rebecca Nieto
- Post Production Manager
- Nathan Gunner
- Compliance Manager
- Linzy Emery
- Development Producer
- Pamela Rosenstein
- Supervising Producer
- Stephen Sweigart
- Business and Production Manager
- Jonathan Loewald
- Senior Producer and Project Director, Margret & Hans Rey / Curious George Producer
- Lisa Mirowitz
- Coordinating Producer
- Laurie Cahalane
- Senior Science Editor
- Evan Hadingham
- Senior Series Producer
- Melanie Wallace
- Executive Producer
- Howard Swartz
- Managing Director
- Alan Ritsko
- Senior Executive Producer
- Paula S. Apsell
Produced by Powderhouse Productions
A NOVA Production by Powderhouse Productions for WGBH
© 2011 WGBH Educational Foundation
All rights reserved
Participants
- Pierre Gygax
- COO, Ulysse Nardin
- Pablo Jarillo-Herrero
- Physicist, MIT
- Jean Lopategui
- Cedars-Sinai Molecular Pathology
- Chad Mirkin
- Institute of Robotics, ETH Zurich
- Daniel S. Mishkin
- Gastroenterologist, Boston University
- Bradley Nelson
- Institute of Robotics, ETH Zurich
- Frances Ross
- IBM Research Division
- Leonie Seliger
- Head of Cathedral Studios
- Stephen L. Smith
- Vice President, Intel
- Mike Tuffey
- Owner, English Antique Glass
- Samuel Wickline
- Cardiologist, Washington University
Education and Outreach Resources
Preview
Full Program | 53:54
Full program available for streaming through
Watch Online
Full program available
Soon