 |
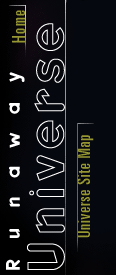 |
|
There are billions of galaxies in the visible universe.
|
How Big is the Universe?
by Brent Tully
How big is the universe? Could it be infinitely large? If the universe has an
edge, what is beyond the edge? And if the universe had a beginning, what was
going on before that?
Our experience of the everyday world does not prepare us to grasp the concept
of an infinite universe. And yet, trying to imagine that the cosmos actually
has a boundary does not make things any easier.
There is an edge to what we are able to see and could ever possibly see
in the universe. Light travels at 300,000 kilometers per second.
That's top speed in this universe—nothing can go faster—but it's relatively slow compared to the distances to be traveled. The
nearest big galaxy to our Milky Way, the Andromeda galaxy, is two million
light-years away. The most distant galaxies we can now see are 10 or 12 billion
light-years away. We could never see a galaxy that is farther away in light
travel time than the universe is old—an estimated 14 billion or so years.
Thus, we are surrounded by a "horizon" that we cannot look beyond—a horizon
set by the distance that light can travel over the age of the universe.
This horizon describes the visible universe—a region some 28 billion
light years in diameter. But what are the horizons of a civilization that
inhabits the most distant galaxies we see? And what about galaxies at the
limits of their vision? There is every reason to think that the universe
extends a long way beyond the part of the universe we can see. In fact, a
variety of observations suggest that our visible patch may be a small fraction—maybe an infinitely small fraction—of the whole universe.
This view of the universe fits with the currently popular idea that the
universe began with a vast expansion of size. The idea describes a kind of
undirected energy present in the vacuum of space, called scalar fields, that
somehow got channeled into a process called "inflation." By conservative
estimates, the universe expanded so much during this period that something the
size of an atom inflated to the size of a galaxy.
If this grand idea is correct, then the universe is larger than we ever could
have imagined. But the question remains: Is there a boundary, and if so, what
lies in the voids beyond? The answer, according to some cosmologists, is truly
mind-boggling. If the universe sprung forth in this manner, then probably
inflation has occurred in other places, perhaps an infinite number of places,
beyond our horizon and outside of our time. The implication is that there are
other universes, perhaps similar to ours or vastly different, each in its own
space and begun in its own time.
The universe began with a vast explosion that generated
space and time.
|
|
Inflation implies a vastly expanded concept of what the universe is. But the
concept is also helping us to understand the universe we see around us. Take,
for example, the recent observation that the universe is not only expanding—a fact astronomers have known for over seven decades—but actually
accelerating outward. That discovery is the subject of NOVA's program "Runaway
Universe."
While we can never directly "see" the whole of the universe or glimpse its
farthest horizons, we can discover how it is behaving—how fast it's growing,
whether its growth will one day come to a halt, and what forces have been
driving its evolution on the largest of scales. The evidence for the cosmic
acceleration—the observations of distant exploding stars called supernovae
(see Birth of a Supernova)—provides a window onto these
behaviors.
The discovery of cosmic acceleration was made by examining the light of
supernovae. We astronomers believe we know the intrinsic brightness of a
particular kind of supernovae, called "Type Ia," so we can calculate how far
such an object must be from us by its apparent, or measured, brightness. We
also know how fast the supernovae—and the galaxies they're in—are rushing
away from us by measuring their "redshift." Redshift refers to a color shift in
the light of galaxies toward the red end of the spectrum as they race away from
us. The faster a galaxy is moving away, the redder its light becomes. (For more
on this phenomenon, go to Moving Targets.)
What we are looking for in this combination of redshift and distance is the
"growth rate" of the universe going back in time. This growth rate tells us
about the gravity of all the matter in the universe—if there is a lot of
matter it will slow down the growth rate over time.
Take the case of a universe with so much matter that gravity arrests the
expansion and everything finally collapses in on itself. We call that a
"closed" universe. In such a universe, the expansion would have once been much
faster. To get to the separations between galaxies that we see now would have
taken a relatively short time. Granted, the numbers associated with "relatively
short" might still seem daunting.
A second possibility might be a universe that is practically empty, often
called an "open" universe. Yes, there must be enough stuff in it to permit the
existence of observers like us, but suppose the total amount of matter has
negligible gravitational influence on the expansion. This universe is just
cruising at the same expansion rate now as in the past. Compared with the first
possibility, the closed universe, expansions in the past would have to have
been slower to get the presently observed separations between galaxies. And it
would mean that a distant supernova observed to be rushing away from us at
such-and-such a speed (redshift) is farther away in this case, compared
to the dense, closed universe case. In the closed universe case, since
expansion was faster in the past, one doesn't have to go so far away (back in
time) to arrive at a specified redshift.
|
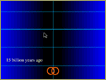
See a comparison between open and closed universes.
Flash required
|
|
So does either of these possibilities describe our universe? No! The one that
comes closest is the "open" universe. However, the supernovae are too faint—that is, they are so far away that even that model doesn't allow the supernovae
to travel as far away as astronomers observe. Our universe, the real one, must
have been loitering after its initial inflationary period, but then put its
foot to the accelerator recently to produce the present separations of galaxies.
Whatever could produce that acceleration? Certainly there is nothing in our
Earthly experience that prepares us for such a possibility. This is where the
theory of inflation comes into play. Now about two decades old, inflation
entertains the idea that there is a kind of energy that causes space to expand.
This energy competes with gravity, though certainly not on local scales.
However, should this form of energy come to dominate, watch out! While gravity
tries to crush, this energy—call it vacuum energy, or the scalar field, or
the energy represented by the Cosmological Constant in Einstein's equations
describing the dynamics of the universe—tries to expand the fabric of space,
pushing everything apart. The basic proposition of the inflation model is that
this form of energy once dominated gravity and caused our universe to burst
forth.
It turns out that the basic inflation picture satisfies a number of observed
facts about the universe. One fact is particularly interesting because the
better our observations become the more tightly they agree with a prediction of
the inflation model. This is that the universe should be "flat"—no overall
curvature of space. Spectacularly convincing evidence—recent measurements of
irregularities in the microwave background radiation—supports this
proposition.
|
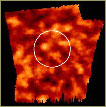
More about experiments on microwave background radiation.
|
|
The microwave radiation comes to us from the time in the past when the universe
was a primordial fireball. We see a "surface" like we see the "surface" of the
sun. We can't look into the sun (or a cloud in the sky) because of scattering
of light. As with the sun and its spots, the surface of last scattering of the
primordial fireball had structure caused by localized regions that were hotter
or cooler, less or more dense. The most pronounced of these structures at the
cosmological surface of last scattering were governed by the distance that
acoustic (pressure) waves could travel in the age of the universe back then,
when the universe was about a half million years old. The size of these
irregularities gives us a ruler! The radiation was emitted so long ago, so far
away, that it has been redshifted down to millimeter wavelengths. So now
millimeter experiments determine the angular size of the clumps caused by
acoustic oscillations in the cooling universe at the surface of the last
scattering.
We know how big the clumps were—a couple hundred thousand light years
across. The relation between their real size, their distance, and the angular
size that we observe is governed by the geometry of the universe. A universe
dense with matter will distort the final size one way, an empty or almost empty
universe will distort another way, and the flat universe of the inflation model
will produce yet a different image, which we would intuitively call
undistorted. Lo and behold, the results are in agreement with the flat
universe of inflation.
This is not the full story. The theory of inflation predicts a precise recipe
of how structure would form from little things merging into big things and
tells us how many little things there should be for each big thing. The
observations match with expectations if the mix of energy and matter is the
same as that suggested by the supernovae experiments. Inflation also solves the
old controversy over the Hubble Constant, the relationship between the rate
galaxies are flying apart and the distances between them. If the Hubble
Constant is large then galaxies are relatively close together and the implied
age of the universe is way too short if the universe has been briskly
expanding. The universe cannot be younger than things in it. However, if the
universe has been loitering and is now accelerating, then it is old enough and
a large Hubble Constant is still possible. And we can actually make a direct
measurement of the mass density of the universe by looking at the motions of
galaxies that slosh in the gravitational wells of the matter. We find something
that has come to be called "dark matter" there. If the universe is "flat,"
then this state is achieved through the sum of the mass and energy density.
Measurements of gravity perturbations reveal just the needed complement of
matter offsetting the repulsive energy indicated by the supernova measurements.
The last couple of years have seen a remarkable convergence of evidence, all
suggesting that we live in a universe with a few percent of the normal matter
of our everyday experience, maybe 25% of something called "dark matter," which
is a name given to hide our ignorance of what it is, and 75% of this energy
that wants to push space apart—call it "dark energy." If true, then
relatively recently in the history of the universe the "dark energy" has become
dominant over "dark matter." During the transient dominance of dark matter, it
caused the collapse into all the structure of the universe that we have come to
know and appreciate.
Maybe we should be less enamored of dark energy. But it is the delight of
physicists because it might provide a laboratory for the moment of creation. It
may be that the present source of repulsion is quite different from the
primordial situation. Certainly the energy density levels and time scales are
vastly different. However, if we can understand the mechanism of the present
acceleration perhaps we can get a clue about the acceleration at the first
instant of our time.
A complicated scenario indeed! So how big is the universe in the inflation
model? It begs the question of what is going on at the boundaries and whether
information could be communicated across universes. We suppose not. It may well
be that only a tiny part of even our own universe is in our horizon, within the
domain that we might hope to know.
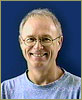 |
|
Brent Tully, an astronomer at the University of Hawaii, works on problems
having to do with the formation of structure and distribution of dark matter in
the universe. The discovery of a tight correlation between the rotation rates
and absolute luminosities of galaxies became known as the "Tully-Fisher"
relation and provides one of the best ways of measuring the size and age of the
universe. Tully was Science Advisor and Co-Producer of the NOVA film "Runaway
Universe." |
Images: (1) NOVA/NCSA; (2) From a simulation of the Formation of Galaxies and Large Scale
Structure by Michael Norman, Brian O'Shea and Greg Bryan, Grand Challenge
Cosmology Consortium (GC3), and visualized by Donna Cox, Stuart Levy, Robert
Patterson, NCSA/UIUC.
History of the Universe |
Birth of a Supernova |
Tour the Universe
Moving Targets |
How Big is the Universe? |
Spin a Spiral Galaxy
Resources |
Transcript |
Site Map |
Runaway Universe Home
Editor's Picks |
Previous Sites |
Join Us/E-mail |
TV/Web Schedule
About NOVA |
Teachers |
Site Map |
Shop |
Jobs |
Search |
To print
PBS Online |
NOVA Online |
WGBH
© | Updated November 2000
|
|
|